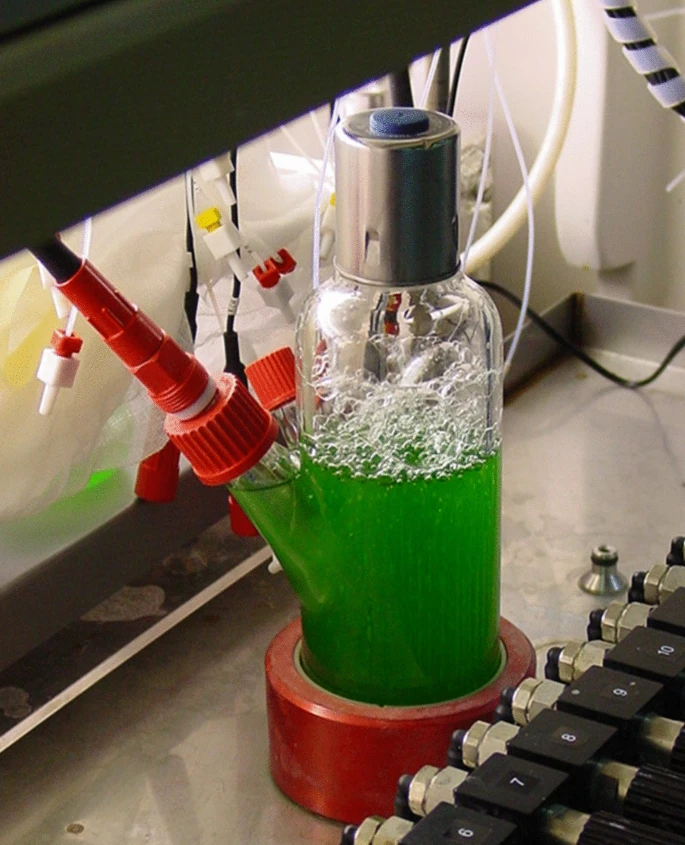
Abstract
Phototrophic microorganisms that convert carbon dioxide are being explored for their capacity to solve different environmental issues and produce bioactive compounds for human therapeutics and as food additives. Full-scale phototrophic cultivation of microalgae and cyanobacteria can be done in open ponds or closed photobioreactor systems, which have a broad range of volumes. This review focuses on laboratory-scale photobioreactors and their different designs. Illuminated microtiter plates and microfluidic devices offer an option for automated high-throughput studies with microalgae. Illuminated shake flasks are used for simple uncontrolled batch studies. The application of illuminated bubble column reactors strongly emphasizes homogenous gas distribution, while illuminated flat plate bioreactors offer high and uniform light input. Illuminated stirred-tank bioreactors facilitate the application of very well-defined reaction conditions. Closed tubular photobioreactors as well as open photobioreactors like small-scale raceway ponds and thin-layer cascades are applied as scale-down models of the respective large-scale bioreactors. A few other less common designs such as illuminated plastic bags or aquarium tanks are also used mainly because of their relatively low cost, but up-scaling of these designs is challenging with additional light-driven issues. Finally, this review covers recommendations on the criteria for photobioreactor selection and operation while up-scaling of phototrophic bioprocesses with microalgae or cyanobacteria.

Introduction
After the earlier ‘green trend’ in the 1980s, bio-based chemicals, such as oleochemicals, gained increasing attention as a sustainable alternative to fossil fuel-based products [1]. The EU Commission identified the chemical industry as one of the closest industries to the EU Green Deal, due to its impact on the end-use sector. The Commission intends to present a chemical strategy for sustainability to protect citizens and the environment from hazardous chemicals, as well as to promote innovation for the development of safe and sustainable alternatives. The priority is to develop new technologies without directly or indirectly using valuable land for plants, food, or fossil fuels [2]. Biotechnological methods can pave the way to more sustainable chemical products of renewable resources [3]. Photosynthetic microorganisms such as microalgae and cyanobacteria have become subjects of interest recently. Converting carbon dioxide provides an environmental advantage over heterotrophic organisms [4], as CO2 sequestration reduces the increase of global warming [5]. Phototrophic microorganisms may become cell factories for the biological synthesis of bioactive compounds. In a study, supported by the European Union, 33 microalgae strains were analyzed as new potential production hosts for active compounds for human therapeutics [6]. Some species of microalgae, particularly brown algae, have also been in the spotlight of food production, thanks to their nutritional value. These organisms contain complex polysaccharides, minerals, proteins, and vitamins as well as diverse phytochemicals, which have interesting therapeutic properties [7]. Bioactives with antioxidative, anti-inflammatory, immunomodulatory, antihypertensive, anticancer, and anticoagulant effects were found in red algae and Spirulina [8,9,10]. With the increasing demand for environmentally friendly alternatives, the development of biofuel and biolubricants has become a common research topic. Lipids produced from microalgae or other microorganisms are the basis for this production [11,12,13]. While plants like rapeseed are nowadays used for lipid production, microalgae seem to be a promising alternative as they do not compete with the food and grow fast [14, 15]. In wastewater treatment applications, mixotrophic microalgae can also be implemented as they use different organic and inorganic substances that would be considered a contaminant otherwise, for their growth [16]. The application of mixotrophic microalgae in combination with wastewater treatment are reviewed in [19]. Aquatic plants and microalgae are also used for phytoremediation, absorbing pollutants such as nitrogen and phosphorus, degrading organic matter, and accumulate heavy metals in their biomass [17, 18].
On an industrial scale, microalgae are usually cultivated in open-pond systems due to the low operational cost; however, the ponds offer an insufficient control of reaction conditions as well as possible contamination from harmful microorganisms [19]. In comparison, closed photobioreactor systems usually offer a higher yield of biomass and product as well as higher photosynthetic efficiency and lower water loss thanks to the controlled environment, but are much more expensive [20].
Ideally, photobioreactors would have a perfect mixing of substrates with little hydrodynamic shear stress for the cells, no dead volume, sufficient gas–liquid mass transfer for CO2 absorption and O2 release, and, in the case of photobioreactors, each microalgae cell would have access to optimal light absorption in any position inside the bioreactor [21]. However, in reality, ideal photobioreactor conditions are impossible to achieve, so different designs of closed photobioreactors are available, each with different techniques to come as close as possible to these ideal conditions to ensure optimal growth and production during the process [19]. In this review, the focus will be on laboratory-scale photobioreactors, which range in volume from a few liters [22] to microliters [23] and are characterized by their use in research instead of the production of consumer goods.
One of the crucial requirements for a photobioreactor is to provide enough light to allow for the growth of the microalgae or cyanobacteria culture. Low light intensities do not deliver enough energy for optimal growth and high light intensities lead to photoinhibition of the algae light-harvesting system [24, 25]. The quantity of light that is emitted by the light source is not the same as what gets absorbed by the photosystems of the cells. Thus, there are different state variables to measure light quantity in the photobioreactor. One is the light intensity. It represents the luminous flux in µmol m−2 s−1 that is irradiated from the light source to the surface of the reactor. There, on the surface, it is also referred to as incident photon flux density. Incident light intensity is easy to measure, can be compared between all different kinds of reactors, and is a basic light parameter mentioned in almost all the literature. Unfortunately, the informative value of the incident photon flux density is limited because light gets attenuated over distance in the suspension as the growing cells absorb the light and shade each other in the culture. Light attenuation should be taken into account. For example, Pfaffinger et al. rely on the mean light intensity that can be calculated depending on the layer thickness of the suspension and biomass concentration of the culture [26].
While on an industrial scale the sun often illuminates the photobioreactors, lab-scale photobioreactors are illuminated by artificial light. It is possible to reach higher biomass or product concentration with the light of a certain wavelength: white, red, and blue light have shown to be sufficient for laboratory-scale cultivation [27, 28]. But because industrial-scale cultivation usually takes place under light-limited conditions, which allow for good process control, growth behavior under a light limitation is also investigated in laboratory photobioreactors [29, 30]. Additional fixtures, that channel the light inside the photobioreactor can enhance light distribution and are easy to scale up by just increasing their number for higher volumes [31, 32]. An alternative to engineering the illumination efficiency of the photobioreactor is to engineer the light-harvesting system of the cultivated microorganism [33]. This can be via genetic modification by reducing chlorophyll b synthesis or red-shifting of the light-harvesting system, for example [34, 35]. Those methods are quite new and still under development, but they have already led to promising results regarding enhanced algae growth under high light intensity or the light of a certain wavelength [36,37,38].
Scaling-up photobioreactor processes present higher complexity compared to conventional reactors, as the heterogeneity of light intensity and availability is a critical factor [39]. Transfer from laboratory to large-scale cultivation of microalgae processes requires careful planning [40, 41]. Besides the crucial light supply, the optimization of state variables such as pH, temperature, CO2 supply and O2 release, inoculum concentration in batch processes and nutrient composition is also required before any scale-up can be considered [39, 42]. Before cultivating at the production scale, the cultivation method needs to be chosen wisely by weighing the different cultivation systems against each other [40]. Scaling factors must be considered from the beginning of the production system and the equipment must be designed accordingly [41]. Lab-scale experiments considering these state variables are imperative to pursue a successful scale-up [39,40,41,42].
Morschett et al. already published a mini review on micro photobioreactors that focuses mainly on microtiter plates, while other reactor types are marginally covered [43]. This review’s claim is to integrate the new insights on microtiter plate cultivation of photosynthetic microorganisms since then and combine them with more detailed information on other laboratory size photobioreactors, to evaluate their advantages and disadvantages in scientific application. Beyond that, this review highlights the potentials of scale-up microalgae cultivation processes based on data generated with the laboratory photobioreactors.

Regarding the scale-up and its concept to a successful industrial application similar review papers also exist [29, 44, 45]. Pruvost et al. discuss parameters to consider when designing and operating microalgal cultivation systems and how appropriate engineering rules can support optimal system design and operation. Moreover, in their review, the focus lies on explaining the influencing parameters to better understand the interrelationship. In previous reviews, the focus is often on the various types of reactors used in industry, their advantages, and disadvantages, and how to handle them. It is often emphasized that the technology transfer from small scale to large scale is essential, but not how and according to which rules this is best approached [39, 46]. On the other hand, most published studies regarding photobioreactors at the laboratory scale only have a very restricted theoretical outlook on scaling up [22, 29, 47]. The technology for the large-scale cultivation of phototrophic microorganisms already exists. Being feasible on this scale is more critical and depends largely on the correct choice of the state variables and the right technology transfer from the laboratory to the industrial scale [48, 49]. The different lab-scale photobioreactors can simulate large-scale conditions in different ways, which is the reason why numerous different reactors exist, all with their special advantages and disadvantages. This review intends to summarize which type of laboratory-scale photobioreactor can simulate large-scale conditions, to what degree, and to give recommendations about which reactor might be used for pre-scale-up experiments. The section on scale-up is intended to provide guidelines based on the existing problems that should be considered during a scale-up.